by Maigha and Andreas Brandt, Commonwealth Edison, USA
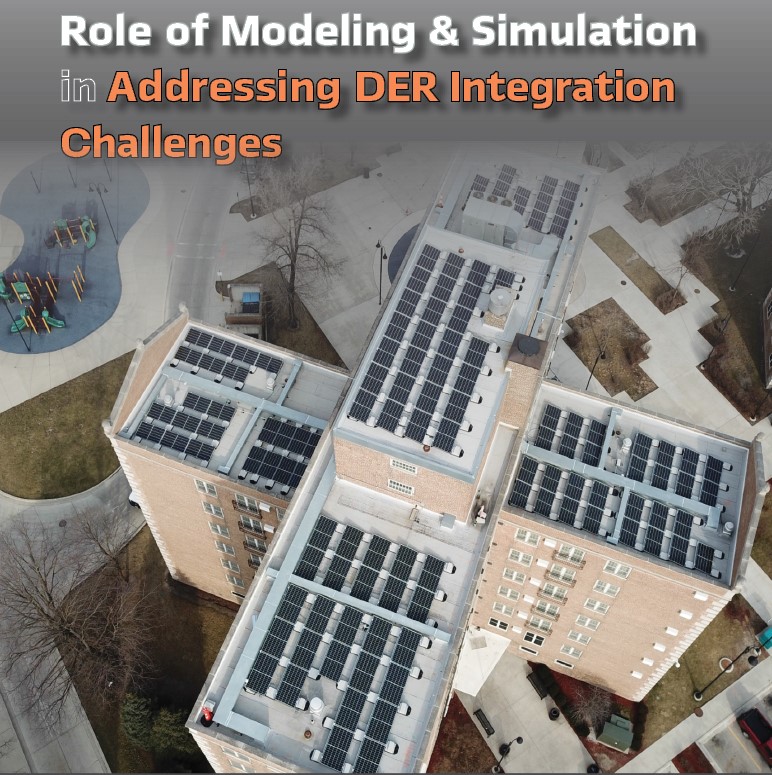
Distributed Energy Resources (DERs) present new challenges to traditional planning and operation philosophies. Well established concepts and theories like ground fault overvoltages, open-phase detection and ferroresonance need revisiting in low inertia grids with inverter-based resources (IBRs). Unlike traditional generation, inverter-based resources present challenges to system protection and operation including their response to system faults, back-feed and voltage/frequency excursions.
What’s more, smart grid technologies coupled with data analytics and artificial intelligence are being used to forward proliferation of DERs in transmission and distribution systems. While bulk generation poses several adequacy, control and reliability challenges on the transmission front, distributed generation and high renewable energy integration have presented unique protection and control challenges at the distribution level. This underlines the importance of using various off-line and real-time modelling and simulation techniques to investigate, support and analyze DER impacts and adaptive protection schemes in a low-risk, low-cost and safer laboratory environment.
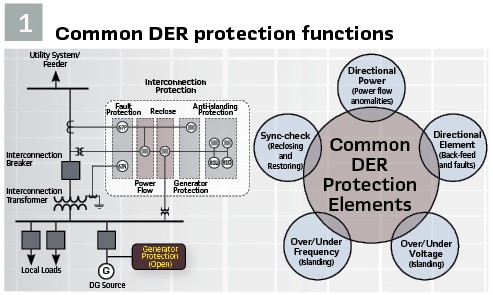
Use of software platforms for modelling system characteristics and studying system phenomena is not new to protection and control. Different aspects like steady state vs. transient simulations, off-line vs. real-time platforms and static vs. quasi-static vs. dynamic paradigms present unique solutions based on the problem being addressed.
Two key components of making detailed investigations include:
1) Selection of right software for the purpose and
2) Model fidelity. Based on the timescales being analyzed, a study may need to evolve from steady state to dynamic simulations.
Recognizing the benefits of these tools, many utilities/electric companies are engaged in utilizing them for not only studying IBR impacts on protection and automation but also researching the applicability of new technologies as mitigation measures through real-time analysis.
DERs and Power System Protection
With greater interconnection, generation being closer to load and increase in power electronics devices like smart inverters, traditional one-way transfer of power is transforming into bi-directional transfer with consumers becoming prosumers. Not only is this mandating a change in markets, stakeholders and policies but also the basic guidelines that made the traditional system design, protection, operation and control reliable and resilient. While some issues may be dealt with using small changes in the existing schemes, others might require development of new techniques, re-visiting design philosophies and control paradigms. Some of the major utility concerns with DER include islanding, sustained overvoltages, exceeding equipment loading limits, and impacts on ground and phase fault current coordination.
Some changes that may result with higher DER penetration are:
- Inclusion of directional element considering reverse fault current
- Addition and coordination of new protection devices or communication schemes
- Changes in current coordination schemes including changing settings and time delays on overcurrent protection.
Conventional Generation vs. Inverter-Based Resource (IBR)
Classical power system has comparable positive and negative sequence impedance. After experiencing short circuit conditions, synchronous generation produces high magnitude current which is dependent on machine parameters, electrical characteristics and impedance of the path to fault. However, current from IBRs has low magnitude due to the thermal limits of power electronic switches. This is controlled by manufacturer specific control algorithms and cannot be universally defined, which contrasts with the physics-driven dynamics of synchronous machines. Inverters operate as current sources with high positive impedance, constant negative impedance and generally do not produce zero sequence currents.
With the inclusion of IBRs, the passive distribution system is transitioning into active sources resulting in a change in the interaction between the transmission and the distribution system. This dynamic behavior of smart inverters needs investigation due to their ability to provide reactive power support, ride through voltage and frequency events and prevent islanding. Additionally, unlike synchronous generators, inverter-based resources (IBR) contribute only 1.1-1.6 pu of their rated current toward faults (low short-circuit contributions). In addition to that, they lack the inertia of a physical generator that poses challenges to frequency stability in low-inertia grids. (Figure 2).
DER Impacts on Protection Coordination
IBRs’ contribution to short-circuit currents is small where their relative penetration with respect to feeder loading is small. Determining this tipping point where their impact becomes important depends on grid characteristics and operating state of the system. Due to the small difference between the normal load current and fault current from an IBR, implementing a reliable, and secure overcurrent protection scheme at PCC is challenging.
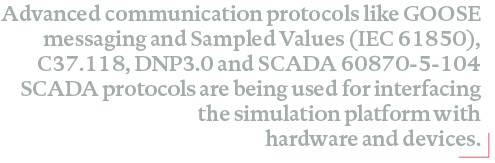
For a downstream fault, the contribution from substation may be reduced due to the short circuit current from DER, thus increasing the trip time for upstream protection device. The effect on protection sensitivity is affected by short circuit strength of the connecting grid, DER type, size and transformer connection, and location of the DER (impedance between DER and substation). This desensitization or blinding effect has been found to be small for high short circuit capacity grids or where DER is located closer to substation. Additionally, under certain conditions specially with rotating DERs, reverse current feeding a fault on a nearby feeder may cause the DER’s feeder relay to misoperate.
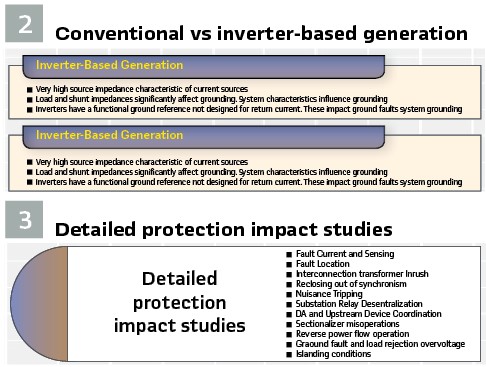
IBR controls are designed to suppress negative sequence currents which may fall to less than 10% of positive sequence current within 1-3 cycles. Therefore, this may not trip negative sequence overcurrent protection. However, with rotating DER and situations with single-phase inverters where generation is close to load, high negative sequence current may result in misoperation of fast-operating, sensitive settings. While rotating DERs may show a consistent relationship between negative sequence current and voltage to determine fault current directionality, this varies in IBRs depending on their negative sequence impedances. This increases the complications with directional settings based on negative sequence measurements.
Interconnection transformers and grounding transformers play an important role in determining ground fault overvoltages on the system with IBRs. While no one configuration is ideal and voltage magnitudes are affected by transformer impedance and the amount of ground-connected load, grounding banks can result in reduced ground fault currents that may affect fault clearance times.
Open phase conditions have been found hard to detect by downstream DERs, especially with the application of ride-through requirements. Manufacturers use proprietary algorithms based on negative sequence components, harmonics or some other power quality metric to sense these conditions that may have results due to fuse blowing or conductor breaking. If inverter controls are found insufficient, feeder-side protection is used to detect and mitigate open phase condition.
Stability of inverter response during disturbances and response during fault recovery may also affect certain system conditions. Typical inverter short circuit current ratio of 2 to 5 is used to ascertain that inverter power output does not change grid voltage and frequency significantly.
With higher penetration, detailed studies may be needed, and a framework needs to be developed to accomplish this within short timelines attributed to interconnection applications. The table below Figure 3 provides a list of studies that may need to be performed for this purpose. The range of events, timescales and tools required for this need further discussion.
DER Modelling, Data Needs & Study Timescales
Depending on the power system event or phenomena being studied, the timescale requirement changes. Figure 4 shows the different timescales for these and identifies the gradation in model complexity as we move from minutes to microseconds. Figure 5 provides an overview of the study needs for steady state and dynamic behavior investigations. Some challenges in adapting effective dynamic models are:
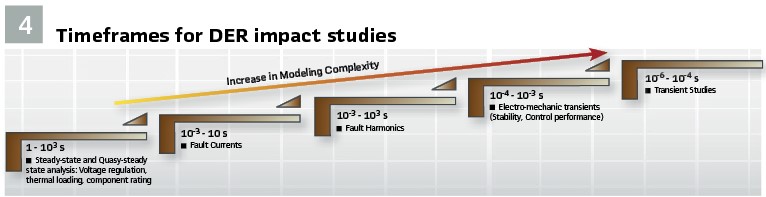
- Model Type: Identification of model details based on event or phenomena under study
- Model Fidelity: Unavailability of validated generic models or detailed manufacturer models
- System Design: Adequate representation of underlying system characteristics
- Integration: Appropriate aggregation demonstrating accurate DER behavior
Steady State vs. EMT Studies: Steady state analysis lays the foundation for most distribution planning applications which captures a particular snapshot in time. When extended to include sequential time-series data with temporal variation in DER inputs, it can be used to run a series of steady state solutions over a window of time (quasi steady-state).
A frequency-domain analysis is used to identify harmonics issues and develop mitigation strategies. Fault analysis programs are generally used to determine device coordination when the system is subjected to fault currents from DER. Grounding practices, interconnection transformers and DER short circuit capabilities are studied to determine any potential issues with conventional protection and coordination schemes. Anti-islanding is another key aspect of this analysis, and such studies need dynamic simulation platforms and models.
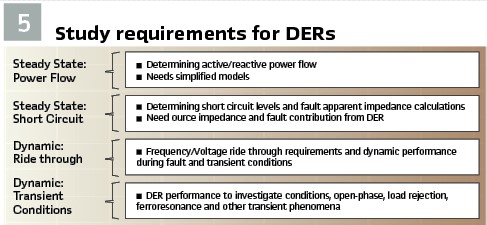
Dynamic studies including electro-mechanical and electro-magnetic studies add to greater complexity in simulation environment. Events that occur in a few milli-seconds to a few seconds timeframe are evaluated. This requires very short simulation times. Electromagnetic transient studies (EMT) are used to study events during the first few cycles of an event like lightning, controlled and uncontrolled switching. Such abnormal conditions, where electrical transients may be present, may affect the system especially with interconnected DER. Some examples include anti-islanding, ride-through during faults, microgrids subjected to disturbances, and DER controls. In contrast to frequency-domain tools, time-domain analysis is needed where all system components are modelled using time-differential equations. Compared to electromechanical transients that have a wider system impact, EMT impacts are more local in nature. Model validation for EMT programs is significantly more challenging than their simplified counterparts in electromechanical programs. Additionally, for an overall reliability impact, deterministic and stochastic tools are used for DER reliability analysis.
Real-time simulations provide unique advantages to enhancing the simulation environment by:
1) Providing real-time analysis using realistic time scales
2) Ability to integrate power and control hardware for device response,
3) Considering communication schemes and time delays
Depending on DER size and utility requirements, reliable two-way communication may be required for protection and control of DERs and utility side devices. With inclusion of fiber for communication and development of advanced protocols like IEC-61850, communication latency and reliability have come to the forefront. Also, communication schemes are not generally modelled in the above-mentioned analysis. Real-time simulations can provide some guidance on latency issues but an open-ended question on co-simulation practices may need to be addressed in the future.
DER Models: Averaged vs. Switched Models: The question that needs to be answered determines the modelling requirements for DER. While simplified models may be adequate for steady state analysis like power flow, planning and short circuit analysis, they may not be enough for evolved dynamic studies. With advanced inverter functions, DERs can no longer be modelled as negative load even in steady state studies. To determine the short circuit contributions for IBRs, their advanced functions, as mandated in interconnection requirements, need to be represented in the steady state planning and short circuit analysis software. To accomplish this, programs use an iterative approach to calculate short circuit contributions.
Particularly for protection related studies such as unintentional islanding, open-phase detection, ground-fault overvoltage, ride-through capabilities, transient stability and fault response EMT models may be best when considering short timescales immediately after disturbance occurs. While average models may be used for the aforementioned phenomenon, frequency stability or long-term voltage stability studies may only need average models. For device coordination and settings, average models of the IBR may be sufficient. Determining which control algorithms need to be implemented also depends on the phenomenon under consideration.
For fault analysis, it may be appropriate to use conservative simple models with appropriate fault current characteristics. Transformer winding configurations, grounding sources and zero-sequence impedance becomes important. Aggregated models may become necessary for representation of three-phase and single-phase DERs. Accurate model of inverter switching, and control requires great expertise and time, which may not be readily available during screening process. Therefore, in the absence of manufacturer data, an IBR may be represented as a voltage controlled current source capable of pushing out a limited fault current during faults.
Advanced smart inverter functions may affect the protection schemes and need to be evaluated in an EMT environment. Due to the variability in proprietary control algorithms for different manufacturers, it is best to use their models for impact evaluations wherever possible.
Model Fidelity: Model validation is critical to identify limitations and confirm model usage scenarios. Offline methods and play-back methods may provide good insights without introducing system-related uncertainties. However, the interaction between DER and system may be missed. Simulating DERs with system model in real time can overcome this shortcoming however, approximations may need to be clearly identified and impact of those on results clearly understood. Model errors may be introduced if incorrect parameters are applied to accurate models or vice-versa.
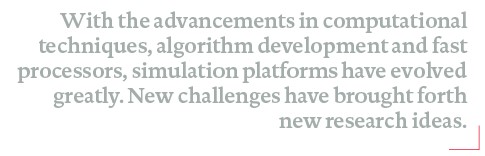
Continuous efforts are being directed toward creating detailed generic models of IBRs however, control algorithms, being manufacturer proprietary, are difficult to replicate and authenticate. This also means that the limitations and approximations made in different models and software need to be well understood.
System Design and Model Aggregation: Depending on the transients or events to be studied, the underlying distribution system model needs to be adapted along with simulation timesteps. For meaningful results, based on the type of study, feeder characteristics, voltage regulation devices, LTCs, interconnection transformers, point of common coupling and system impedance may need to be modelled at varied granularity levels. Furthermore, temporal variation in DER output needs to be modelled in significant detail based on application. Apart from manufacturer equipment data and inverter models, other data sources that can be explored are: utility historical data, load measurements from AMI or SCADA, PV generation measurement, event logs from DA devices and relays, and inverter event logs and power production.
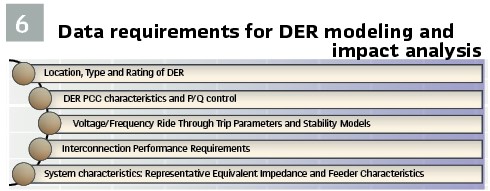
Model complexity also results in computational overhead, thus limiting the simulated system size. In some cases, reduced model of the selected system is used to avoid the exponential growth in computational complexity. Hence, base model needs to be tested for accuracy to authenticate study results. (Figure 6).
DER Simulation Guidelines
In protection and control, simulations have been used to test coordination strategy and determine settings using short-circuit programs. Some aspects also used arc-flash, and other transient phenomena to determine safe limits of operation. As explained in the sections above, the question under consideration determines the type of study, timescales, and tools. Therefore, while real-time simulation results may seem closest to practical implementations, given the prohibitive costs, stability issues and model limitations, they may not be the best option for studies.
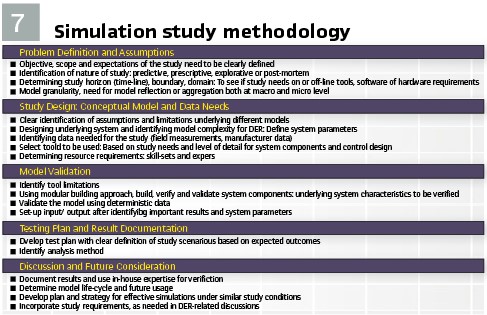
A basic framework for designing DER studies presented in Figure 7 outlines a methodology adapted to show the progression, provide checking points and provide an overarching overview of the study process for DER protection studies.
Application of Data, Artificial Intelligence/Machine Learning to Protection
Data driven business analytics, operations and control have found applications in the field of power systems recently. Some of the artificial intelligence (AI) techniques include artificial neural networks, fuzzy logic, expert systems and genetic algorithm and can be broadly classified into four categories: 1) supervised learning, 2) unsupervised learning, 3) semi-supervised learning and 4) reinforcement learning. Some of the potential applications in power systems include forecasting, load prediction, anomaly detection, phasor measurement unit (PMU) data interpretation, equipment failure, and large-scale optimization. The area of protection has seen some limited applications due to the critical nature of protection coordination in safe and reliable system operation. They have been investigated and researched upon for development of adaptive schemes, however, these suggestions lack practical implementation maturity.
In practice, relays use system conditions and a set of rules to classify an abnormal situation. The key question or consideration in applying AI to drive relay operations is to understand the efficacy of data-driven operations compared to physics-based response of protection devices. For a system where misoperations and nuisance tripping are part and parcel of a 99.9% reliable system, some key challenges associated with data or AI based applications include:
- Scalability of AI methods while maintaining reliability
- Unavailability of granular and high-fidelity training data
- Limitations on implemented settings based on available data, data resolution and resulting measurement and computation errors
- System conditions and configurations change which requires adaptability
- Flexibility of one design to be implemented to another feeder design or configuration
- Lack of information on complete protection design including primary and backup
- Ascertaining success of an AI based technique and trouble-shooting any discrepancies
AI methods may be most suitable in applications that either do not need physics-based decision-making or those to which physics-based protection philosophies are not applicable e.g., detecting misoperations and incipient faults. Techniques that do not heavily rely upon training data may also be good candidates for AI applications. These may use physics-aware methods and interpretable AI.
Conclusion
With the advancements in computational techniques, algorithm development and fast processors, simulation platforms have evolved greatly. New challenges have brought forth new research ideas. Some examples include estimation-based protection that explores the concept of setting-less relay, travelling wave protection, and model-driven adaptive protection Real-time simulation platforms like RTDS are being used extensively in industry and academic environments for hardware-in-the-loop testing of state-of-the-art concepts and devices.
With the provision of massive data facilitated by advanced measurement and sensing devices coupled with fast communications in DER proliferated distribution systems, robust solutions are being sought. As this domain evolves, simulations will continue to be used not only for coordination studies, impact analysis, event analysis, and settings development but also for automated protection modelling, fault location, communications testing, and compliance studies. In fact, co-simulation of protection studies with communication layer and cyber-security layer will be deemed necessary as more DERs are integrated, thus marking a shift in the traditional power system operation and control paradigm.
Biographies:

Maigha a licensed professional engineer, received her Ph.D. in Electrical Engineering at Missouri University of Science and Technology, Rolla, MO followed by a post-Doctoral research fellow position at Clemson University Restoration Institute, Charleston, SC. She is currently serving as a Senior Engineer in the Relay and Protection Engineering department at Commonwealth Edison, Illinois, USA. In her current role she is working on developing simulation and analysis framework for assessing DER impacts on the distribution system. She is a senior member of IEEE and is engaged with CIGRE – Women in Energy. Her research interests include DERs, smart inverters, EVs, microgrids, real-time simulations and hardware-in-loop testing.

Andreas Brandt is the manager of Distribution Protection and Modernization group within Relay and Protection department at Commonwealth Edison, a leading utility in IL, USA. An engineer by training, he has more than 20 years of experience in the utility industry. Brandt holds a MS degree in electrical engineering from Illinois Institute of Technology and is a registered professional engineer. He is leading the efforts to address protection issues with increasing distributed energy resource penetration.